Antidepressant, neuropharmacological activity and mode of action of theaflavin-3-gallate in in vitro and in vivo models of depression
Abstract
Herein the antidepressant-like effect of theaflavin-3-gallate, a plant polyphenolic compound in 1-methyl-4-phenyl pyridinium-induced neuronal injury in N2a cells used as an in vitro model of depression as well as in vivo. The results from MTT (3-(4, 5-dimethylthiazol-2-yl)-2, 5-diphenyltetrazolium bromide) and lactic dehydrogenase assay revealed that theaflavin-3-gallate exerted a neuroprotective effect in N2a cells. Treatments with mixtures of 1-methyl-4-phenyl pyridinium (20 µM) and four concentration levels of theaflavin-3-gallate increased cell viability from 23.3 to 79.6% while lactic dehydrogenase leakage value decreased from 63.2 to 23.4 U/L. Fura-2-acetoxymethyl ester assay for Ca2+ concentration measurement revealed that different doses of theaflavin-3-gallate or fluoxetine (20 µM) reduced the intracellular Ca2+ overloading in N2a cells induced by 1-methyl-4-phenyl pyridinium. Inverted phase microscopy showed that theaflavin-3-gallate induced neurite outgrowth in 1-methyl-4-phenyl pyridinium-induced neurotoxicity in N2a cells. Theaflavin-3-gallate could significantly reduce the immobility time of mice in both forced swimming test and tail suspension test.
Introduction
Depression is one of the major, life-threatening psychiatric disorders associated with symptoms such as consistent negative moods, reduced physical activities, feelings of helplessness, and lethargic thought and cerebral function. Depression causes a considerable burden on health worldwide and remains a challenge because of inadequate treatment.
Stressful environment, hostile life-event and lack of supporting relationship are several of the contributing factors that contribute to the advancement of depression in humans (Bhutani et al., 2009; Baum et al., 2004). The irregularities of noradrenergic, dopaminergic and serotonergic transmission are known to cause mental depression (Berton and Nestler, 2006).
Growing evidence suggests that inflammatory processes play a key role in the pathophysiology of major depressive disorder. In major depressive disorder patients, there are irregularities in synaptic and structural plasticity in different locations of the brain, including the frontal cortex and hippocampus (Rajkowska, 2000; Cotter et al., 2001; Cotter et al., 2002). These irregularities were thought to be accountable for the failure of brain to react appropriately to environmental stimuli (Duman, 2002). Consequently researchers worldwide started to study the effect of neurotrophins in depression because they are of critical importance in establishment and maintenance of neural circuits (Oliveira et al., 2013; Callaghan and Kelly, 2013). Multifarious lines of evidence associate brain-derived neurotrophic factor, its specific receptor tropomyosin-receptor-kinase B (TrkB) to the pathophysiology of major depressive disorder, as well as the therapeutic mechanisms of antidepressants. Down-regulation of brain-derived nerve growth factor expression in the dentate gyrus and hippocampus of rats on chronic stress indicated the potential contribution of brain-derived nerve growth factor in depression (Lee and Kim, 2010).
The treatment regimen for depression typically consists of moclobemide (a selective and reversible monoamine oxidase inhibitor), citalopram (selective serotonin reuptake inhibitor) and venlafaxine (serotonin and nor-adrenaline reuptake inhibitor). However, the majority of these drugs are synthetic compounds which often possess adverse effects especially cardiovascular problems (Xu et al., 2004). Hence, there is a pressing requirement for the design and development of more efficacious antidepressants with little or no adverse effects. Herbal medicines may provide the alternative, cheap and relatively safe antidepressants. Recently, more herbal medicine has been used as alternative therapy for depression. Several Chinese medicinal plants have been effectively used to treat sentimental diseases, which is similar to depression in Western medicine. Furthermore, the bioactive principles from them were also extracted and isolated (Yu et al., 2002; Rocha et al., 2007).
Many plants or their products have been suggested to have antidepressant and antianxiety effects including Hypericum perforatum (Ernst, 2007; Mills et al., 2005), Ziziphus spina-christi (Setorki and Hooshmandi, 2017), Glycyrrhiza glabra (Muralidharan et al., 2008), Hyssopus officinalis (Salehi and Setorki, 2017), Mentha pulegium (Rabiei et al., 2016), Nyctanthes arbor-tristis (Gupta et al., 2016), Piper methysticum (kava) (Pittler and Ernst, 2003), Sambucus ebulus (Mahmoudi et al., 2014) and Sambucus nigra (Mahmoudi et al., 2014).
The objective of the present research work was to investigate the antidepressant, neuropharmacological activity along with the mode of action of theaflavin-3-gallate, a plant polyphenol in in vitro and in vivo models of depression.
Materials and Methods
Reagents
Theaflavin-3-gallate (purity >98%), 1-methyl-4-phenyl pyridinium and fluoxetine (purity >98%) were purchased from the Sigma-Aldrich (USA). Dulbecco’s modified Eagle’s medium culture medium, trizol reagent, fetal bovine serum, heat-inactivated horse serum, penicillin and streptomycin were purchased from the Gibco BRL (USA). 3-(4, 5-Dimethylthiazol-2-yl)-2,5-diphenyl tetrazolium bromide (MTT) was purchased from the Merck Chemical Co. (USA). All other chemicals and reagents were of analytical grade unless otherwise specified.
Cell line and culture conditions
The N2a cell line (fast-growing mouse neuroblastoma cell line) was used to study the neuroprotective effect of theaflavin-3-gallate. The cell line was maintained in Dulbecco’s modified Eagle’s medium culture medium supplemented with 10% fetal bovine serum (FBS), 2 mM l-glutamine, 100 U/mL penicillin, and 100 µg/mL streptomycin at 37°C in 5% CO2 in a humidified atmosphere. Differentiation was induced by removing the growth medium and then replacing with an equal volume of Dulbecco’s modified Eagle’s medium culture medium 3% FBS, 1.5 mM l-glutamine, 100 U/mL penicillin, 100 µg/mL streptomycin, and 10 µM all-trans retinoic acid.
Box 1: MTT Assay
Principle
There is conversion of MTT to formazan crystals within the living cells (culture cell line), which determines the mitochondrial activity. It is not possible to calculate the mitochondrial activity of single cell. That is why, the total mitochondrial activity of only viable cells are measured. The viable cells have NAD(P)H-dependent oxidoreductase which reduces the yellow colored MTT reagent to deep purple colored formazan (an insoluble crystal). The darker the solution is due to the greater number of viable and metabolically active cells.
Requirements
MTT (3-[4,5-dimethylthiazol-2-yl]-2,5 diphenyl tetrazolium bromide); Plateshaker; Singla channel pipette (0.001–1 mL); Multichannel pipette (0.01–0.3); Class 2B hood Benchtop centrifuge; Microplate reader (Thermo Molecular Devices Co., USA); Incubator with 5% CO2 at 37°C; Microplates-96 well; round or flat bottom well; Magnetic stirrer; Filter (0.22 mm); Methanol, ethanol, and dimethyl sulfoxide; Formazan; Neuro2a cells (Shanghai Institute of Cell Biology, Chinese Academy of Sciences (China); Dulbecco’s modified Eagle’s medium culture (serum-free low sugar)
Procedure
Preparation of MTT solution
Step 1: Dissolve MTT powder (500 mg) in phosphate buffer solution (5% solution; 10 mL)
Step 2: Use a magnetic stirrer to stir the solution for approximately 1 hour in the dark
Step 3: Use a filter (0.22 mm) to sterilize the solution and store in 10-mL aliquot at -20°C
Step 4: Unused MTT may be frozen and reused
Incubation of cells
Day 1
Step 1: Add Neuro2a cells into the 5 mL Dulbecco’s modified Eagle’s medium culture
Step 2: Centrifuge the medium in a sterile falcon tube (15 mL) at 500 rpm for 5 min
Step 3: Remove the media and resuspend cells to culture medium (1.0 mL)
Step 4: Count and record cells per mL aseptically
Step 5: Dilute the cells to 106 cells/mL. Use the Dulbecco’s modified Eagle’s medium to dilute cells
Step 6: Add 100 µL of cells (105 total cells) into each well and incubate for 48 hours
Day 3
Step 1: When removing the media, do it carefully in order to avoid variation in data
Step 2: Final volume should be 100 µL/well
Step 3: Treat the cells with a) no drug (untreated control); b) 1-methyl-4-phenyl pyridinium (20 µM); c) 1-methyl-4-phenyl pyridinium (20 µM) plus fluoxetine (20 µM); d) 1-methyl-4-phenyl pyridinium (20 µM) plus theaflavin-3-gallate (5 µM); e) 1-methyl-4-phenyl pyridinium (20 µM) plus theaflavin-3-gallate (10 µM); f) 1-methyl-4-phenyl pyridinium (20 µM) plus theaflavin-3-gallate (50 µM); g) 1-methyl-4-phenyl pyridinium (20 µM) plus theaflavin-3-gallate (100 µM)
Step 4: Cells are plated in triplicate to minimize the variation in results. Incubate the cells for 48 hours
Day 6
Step 1: Remove the medium and wash cells with phosphate buffer solution
Step 2: Add 20 µL of 1 mg/mL MTT to each well. All should be done aseptically
Step 3: Use a plateshaker to shake the plate for 5 min (slowly increasing the shaking speed: maximum 900 shakes/min)
Step 4: Then incubate the plate in a CO2 incubator for another 3 hours at 37°C, depending on the cell type until the intracellular formazan crystals are visible under a microscope
Step 5: Dimethyl sulfoxide (5%, 100 µL) is added to each well and resuspend until all crystals have been dissolved
Step 6: Using a multichannel pipette to mix each well thoroughly
Step 7: Measure the absorbance at 540 nm using the microplate reader
Precautions
MTT is toxic and harmful. There may be irritation of the skin and eye. Therefore, use equipment for personal protection
MTT is light sensitive. The bottle containing MTT solution should be covered with aluminum foil to protect from light. It is necessary to check the color of the MTT stock solution before use. If it is green or blue in color instead of yellow, then one can think that the stock solution has either been contaminated by bacteria or light exposer. If it looks properly, then store at 4°C. The stock solution of MTT can be used up to 18 months.
Always include a blank (well containing the medium only) and a positive control (untreated cells) and plate these samples in triplicate.
Precipitate may form when serum or albumin contain high amount of protein in the culture medium is added to the MTT solution. The protein concentration equivalent to 10% fetal bovine serum is acceptable.
Always wash cells with the phosphate buffer solution (step 2) before adding the MTT in order to remove cellular debris or dead cells that can give inaccurate result.
The percentage of dimethyl sulfoxide should not be more than 10%
Limitations of the assay
The sensitivity of MTT assay is lower than that of luminescent or fluorescent assay in case of cells that with low metabolic activity or cells that do not readily proliferate.
Some chemicals like polyphenols, vitamin A, coenzyme A, and dithiothreitol interfere with the reduction of MTT to formazan and are not easily compatible with the MTT assay. Both exposure to the MTT solution and the formation of formazan crystals are cytotoxic and may damage the cellular morphology.
Absorbance values
The absorbance readings should be within 0.75 and 1.25. If the reading is too low, then increase the number of cells plated or the incubation time, and make sure that the cell culture condition is appropriate. Plating too many cells/well will yield high absorbance, as will contamination by the yeast or bacteria.
The optimal number of cells per well is important. For this reason, prepare and plate serial dilutions of cells in the medium from 103 to 106 cells/mL. This will help to decide the number of cells to obtain the appropriate absorbance value.
References
Slater et al., 1963; van de Loosdrecht et al., 1991; Alley et al., 1988; Kou et al., 2017; Bahuguna et al., 2017
Cell viability evaluation by lactic dehydrogenase release assay
Six groups of N2a cells were used as per the following protocol: Untreated control (no drug), 20 µM 1-methyl-4-phenyl pyridinium, 20 µM 1-methyl-4-phenyl pyridinium plus 20 µM fluoxetine, and 20 µM 1-methyl-4-phenyl pyridinium plus theaflavin-3-gallate (5, 10, 50 and 100 µM). The cells were seeded in 96-well culture plate at a density of 1 × 105 cells/well (n=6, for each group with equal cells). The cells were incubated with the desired drugs (or no drug in case of control) in serum-free low glucose DMEM for 48 hours. The medium was then collected and lactic dehydrogenase activity was determined with a lactic dehydrogenase assay kit (Roche Diagnostics GmbH, Germany). Following 30 min at room temperature in the dark, the absorbance was recorded on a microplate reader at a wavelength of 490 nm and the lactic dehydrogenase activity was calculated from the decrease of NADH absorbance resulting from the conversion of pyruvate to lactate.
Measurement of intracellular Ca2+ concentration
The protocol for the determination of Ca2+ was used in this study (Li et al., 2004). N2a cells were seeded onto 24-well plates at a density of 2 x 106 cells/well. The cells were given treatment as follows: Untreated control (no drug), 20 µM 1-methyl-4-phenyl pyridinium, 20 µM 1-methyl-4-phenyl pyridinium plus 20 µM fluoxetine and 20 µM -methyl-4-phenyl pyridinium plus theaflavin-3-gallate (5, 10, 50 and 100 µM). At the end of the treatment, the cells in each group were collected and then incubated with the complete medium containing 10 M Fura-2-acetoxymethyl ester at 37°C for 50 min. Afterward, the cells were washed twice and resuspended with cold phosphate buffer solution containing 0.3% bovine serum albumin. After incubating for another 10 min at 37°C, the concentration of Ca2+ was determined by recording emission changes at 510 nm with an excitation shift from 340 nm to 380 nm, using a fluorescence spectrophotometer.
Determination of neurite outgrowth
The N2a cells were stained with crystal violet for calculating the number of cells showing neurites along with measuring the neurite length. The cell cultures treated with or without the theaflavin-3-gallate (5, 10, 50 and 100 µM) in 6-well plates were washed with phosphate buffer solution before fixing with ice-cold methanol at –20°C for 20 min. The cells were then stained with 0.8% crystal violet solution in methanol for 20 min. By means of an inverted light microscope at 400x magnification, we counted the percentage of cells expressing neurites and measured average neurite length. Six random fields were examined from each well, giving a total cell count of at least 300 cells/well. Each data point symbolizes the mean of three individual wells in one experiment, and each experiment was repeated three times.
Animals
Male Sprague-Dawley rats were obtained from the Laboratory Animal Unit of Zhejiang Chinese Medicine University (China). The animals weighed 170 ± 10 g and were acclimatized to the housing facilities before 7 days of use in the experiments. The animals were housed 5 per cage and acclimatized to a colony room with controlled ambient temperature (25 ± 2°C), humidity (45 ± 5%) and a 12 hours light/dark cycle. The rats were fed a standard diet and had free access to water.
Drugs administration
The animals were randomly assigned into control and six experimental groups (8 mice per group) as follows: vehicle-treated; Theaflavin-3-gallate 20 mg/kg; Theaflavin-3-gallate 40 mg/kg; Theaflavin-3-gallate80 mg/kg; fluoxetine 20 mg/kg; amitriptyline 20 mg/kg. Theaflavin-3-gallate and all drugs were intraperitoneally administered once. Theaflavin-3-gallate was dissolved in 10% Tween-80 and diluted to the desired concentration on the day of testing while fluoxetine and amitriptyline were dissolved in normal saline (0.5% NaCl). Vehicle and theaflavin-3-gallate were injected intraperitoneally 20 min before testing. Fluoxetine and amitriptyline were administered through the same route.
Forced swimming test
The forced swimming test is the most widely used pharmacological in vivo model for assessing the antidepressant activity. The development of immobility when mice are placed in an inescapable cylinder filled with water reflects the cessation of persistent escape-directed behavior (Porsolt et al., 1977). Briefly, mice were individually forced to swim in an open cylindrical container (diameter 12 cm, height 24 cm), with a depth of 16 cm of water at 25 ± 1°C. The immobility time, defined as the absence of escape-oriented behaviors, such as swimming, was scored during 8 min with the help of stopwatch, as defined previously (Eckeli et al., 2000; Zomkowsi et al., 2004). Each mouse was adjudged to be immobile when it stopped struggling and remained floating stationery in the water, making only those movements necessary to keep its head above water. The parameter obtained was the number of seconds spent immobile. The water in the containers was changed after each trial.
Tail suspension test (Steru et al., 1985)
Mice both acoustically and visually isolated were suspended 50 cm above the floor by adhesive tape placed approximately 1 cm from the tip of the tail. The total immobility period was scored manually during 8 min test session with the help of stopwatch. Rats were considered immobile only when they hung passively and completely motionless. The test was recorded using videotape in a dark room with minimum background noise. The parameter obtained was the number of seconds spent immobile.
Open field test
The open field area was comprised of transparent acrylic walls and a black floor (50 × 50 × 25 cm) divided into nine squares of equal size. One hour before the test, the mice were treated with drugs as described. The open field test was used to evaluate the locomotor activity of mice that had previously been subjected to the forced swimming test. The experiential parameters included the number of squares crossed (with four paws) and the number of rearings. The number of squares crossed with all paws (crossing) were observed and counted in 60 min. During the test session, the crossing activity of mice was recorded and saved using a video camera for the permanent record (Moklas et al., 2008). The square arena was cleaned with a solution of 10%alcohol between tests and dried after occupancy by each mouse in order to hide animal clues and to prevent each mouse from being influenced by the odors present in the urine and feces of the previous mouse.
Results
Effect on N2a cell viability after 1-methyl-4-phenyl pyridinium treatment
The viability of N2a cells exposed to 1-methyl-4-phenyl pyridinium (20 µM) for 48 hours was considerably diminished in comparison to the control group, with a survival rate of 11.5% of the control (Figure 1). When N2a cells were treated with a mixture of 1-methyl-4-phenyl pyridinium (20 µM) and fluoxetine (20 µM), the cell viability was significantly increased as compared to the 1-methyl-4-phenyl pyridinium group. Treatments with mixtures of 1-methyl-4-phenyl pyridinium (20 µM) and four concentration levels of theaflavin-3-gallate (5, 10, 50 and 100 µM), the cell viability was increased in a dose-dependent manner as compared to the 1-methyl-4-phenyl pyridinium treatment. The survival rates for these treatments were 67.1% (fluoxetine), 23.3, 39.4, 63.1 and 79.6% respectively (Figure 1).
Figure 1: Effect of theaflavin-3-gallate on cell viability and LDH leakage in 1-methyl-4-phenyl pyridinium (MPP)-treated N2a cells. Values given are the mean ± S.E.M. (n = 6). ap<0.01 as compared with control group; bp<0.05, cp<0.01 as compared with 1-methyl-4-phenyl pyridinium group; Contr means control; FLU means fluoxetine
Effect on lactic dehydrogenase release in 1-methyl-4-phenyl pyridinium-treated N2a cells
After treatment of N2a cells with 1-methyl-4-phenyl pyridinium (20 µM) for 48 hours, lactic dehydrogenase leakage was 75.2 U/L as contrasted to 8.4 U/L in the control group (Figure 1). When treated with 1-methyl-4-phenyl pyridinium (20 µM) along with fluoxetine (20 µM) or with theaflavin-3-gallate (5, 10, 50 and 100 µM), lactic dehydrogenase leakage values were 28.5, 63.2, 48.2, 35.6 and 23.4 U/L, respectively, all much lower than with 1-methyl-4-phenyl pyridinium alone, although the effect of 1-methyl-4-phenyl pyridinium was not totally upturned (Figure 2). Thus, theaflavin-3-gallate inhibited the lactic dehydrogenase release from the N2a cells in a dose-dependent manner.
Figure 2: Effect of theaflavin-3-gallate on the concentration of Ca2+ ion in 1-methyl-4-phenyl pyridinium-treated N2a cells. Values given are the mean ± S.E.M. (n = 6). ap<0.01 as compared with control group; bp<0.05, cp<0.01 as compared with 1-methyl-4-phenyl pyridinium group
Effect on Ca2+ concentration of 1-methyl-4-phenyl pyridinium-treated N2a cells
Calcium is a critical factor for the development of neurons and intracellular calcium overloading may result in neuronal injury (Zomkowsi et al., 2004). Therefore, determining calcium concentration is an indication of neuronal injury and toxicity. Treatment of N2a cells with 1-methyl-4-phenyl pyridinium (20 µM) for 48 hours caused a substantial increase in Ca2+ concentration over the control group (842.2 vs. 320.5 nmol/L) (Figure 2). On the other hand, in treatments with 1-methyl-4-phenyl pyridinium (20 µM) along with fluoxetine (20 µM) or with theaflavin-3-gallate (5, 10, 50 and 100 µM), Ca2+ concentrations were considerably lower (being 487.2, 570.8, 510.5, 465.2 and 410.6 nmol/L, respectively; p<0.05) than with 1-methyl-4-phenyl pyridinium alone (842.2 nmol/L).
Theaflavin-3-gallate promotes neurite outgrowth
To determine the effect of theaflavin-3-gallate exposure on neuronal differentiation, we treated differentiating N2a cells with various concentrations of theaflavin-3-gallate (5, 10, 50 and 100 μM) for 48 hours. As shown in Figure 3, retinoic acid treatment led to apparent changes in neuronal cell morphology like shrinkage of the cell body and neurite outgrowth. Treatment with 1-methyl-4-phenyl pyridinium (20 µM) exhibited an inhibitory effect on neurite outgrowth and the number of cells with decreased neurite length increased. However, on treating with different doses (5, 10, 50 and 100 μM) of theaflavin-3-gallate for 48 hours, showed a reverse effect with the number of neuronal cells showing neurite outgrowth increasing with increasing dose of theaflavin-3-gallate.
Figure 3: Effect of various doses of theaflavin-3-gallate on the neurite outgrowth of N2a cells measured by inverted light microscope (400x magnification at retinoic acid treated cells (A); 1-methyl-4-phenyl pyridinium (20 µM) treated cells (B); 5, 10, 50 and 100 µM theaflavin-3-gallate-treated cells (C-F) respectively. The neurite outgrowth can be clearly seen in theaflavin-3-gallate-treated cells
Effect on immobility time of forced swimming test
Theaflavin-3-gallate induced a significant antidepressant effect in the forced swimming test because it considerably reduced the immobility time compared with the control. After 40 min of treatment, theaflavin-3-gallate substantially decreased the duration of immobility time at 20, 40 and 80 mg/kg in comparison to control. Antidepressant positive control drugs fluoxetine (10 mg/kg) and imipramine hydrochloride (10 mg/kg) caused a considerable reduction in the immobility time of mice forced swim test as compared to control (Figure 4).
Figure 4: Effects of theaflavin-3-gallate (20, 40 and 80 mg/kg), fluoxetine (10 mg/kg) and imipramine hydrochloride (10 mg/kg) on immobility time in the mouse forced swim test (A), immobility time in the mouse tail suspension test (B) and number of crossings in open-field tests (C). Theaflavin-3-gallate was administered intraperitoneally 30 min before the test. Each column represents the mean ± S.E.M. of immobility period (s) of 8 rats in each group (n = 8). ap<0.05 compared to control; bp<0.01
Effect on immobility time of tail suspension test
Theaflavin-3-gallate (20, 40 and 80 mg/kg) significantly and dose-dependently decreased (p<0.05) immobility time of mice in tail suspension test in comparison to the control. The antidepressant positive control drugs used that is fluoxetine (10 mg/kg) and imipramine hydrochloride (10 mg/kg) caused a considerable reduction (p<0.05) of immobility time as compared with control. Figure 4 shows the effect of theaflavin-3-gallateon immobility time in the mouse tail suspension test.
Effect on locomotor activity of open field test
Theaflavin-3-gallate only at a higher dose (80 mg/kg) slightly increased (p<0.05) the number of crossing activity of mice in open field test as compared to the control, the lower doses of it were ineffective in producing this effect. Interestingly, the two antidepressant positive control drugs viz., fluoxetine (10 mg/kg) and imipramine hydrochloride (10 mg/kg) also produced a lower effect. Figure 4 shows the effect of theaflavin-3-gallate on the number of crossings in open-field test within 60 min.
Discussion
Various polyphenolic compounds have been shown to exert potent effects on alleviating inflammatory diseases and neural degeneration (Kim and Yoon, 1998). Studies have revealed the protective effect of these polyphenols in different neurological and mental disorders. These polyphenols modulate monoaminergic neurotransmission in the brain and thus exhibit antidepressant activity in in vitro and in vivo models of depression. Curcumin, apigenin, chlorogenic acid, amentoflavone, ellagic acid, hesperidin, naringenin and proanthocyanidins are among the polyphenols which have been reported to show anti-depressant activity in animal models of depression. Various mechanisms have been put forward to explain the antidepressant potential of these molecules. These include modulation of various neurotransmitters such as norepinephrine, serotonin and dopamine. Antioxidant, anti-inflammatory properties of these molecules also have been suggested to explain their antidepressant effect. The polyphenols also modulate the activity of MAO enzyme and they may also act as neurotransmitters (Pan et al., 2010; Pathak et al., 2013).
Theaflavin-3-gallate is a polyphenolic compound in black tea, formed by oxidation and polymerization of catechins during tea fermentation process. Theaflavin-3-gallate is an antioxidant as effective as the catechin of green tea and is the most potent antioxidant and bioactive compound among black tea theaflavin derivatives. Theaflavin-3-gallate has also been shown to be effective in anti-obesity, anti-inflammation, cancer chemoprevention, fatty liver prevention, and improvement of insulin sensitivity (Butterweck et al., 2000; Leung et al., 2001).
In the present study, we evaluated the in vitro as well as the in vivo antidepressant activity of theaflavin-3-gallate. We studied the possible cellular mechanisms of theaflavin-3-gallate in 1-methyl-4-phenyl pyridinium -induced neurotoxicity using N2a cells as a suitable in vitro model of depression. After treating N2a cells with 20 µM 1-methyl-4-phenyl pyridinium for 48 hours, the viability of the cells was dramatically decreased while as the LDH leakage was significant as compared to the untreated control cells pointing to the fact that the N2a cells were physiologically damaged and even many of the cells were dead. However, on treatment with theaflavin-3-gallate, the viability of the cells was increased and the LDH leakage was less. This result suggests that theaflavin-3-gallate has the ability to partly reverse the toxic effect of 1-methyl-4-phenyl pyridinium. Theaflavin-3-gallate has the tendency to enhance the viability of N2a neurons by protecting them from 1-methyl-4-phenyl pyridinium-induced injury. We also evaluated the effect of theaflavin-3-gallate on the Ca2+ concentration in these cells. Many published reports reveal that calcium, which is a key element for the neuron development, its overloading may led the neuronal injury. In this study, we observed that 20 µM 1-methyl-4-phenyl pyridinium caused Ca2+ overloading which was significantly and dose-dependently reduced after theaflavin-3-gallate treatment. Thus, theaflavin-3-gallate show the neuroprotective effect by suppressing Ca2+ overloading. Theaflavin-3-gallate also induced significant neurite outgrowth in N2a cells after initial exposure to 1-methyl-4-phenyl pyridinium (20 µM).
In vivo results showed that theaflavin-3-gallate showed statistically significant results in Forced swimming test (reduction in immobility time) with treatment of theaflavin-3-gallate at various doses. The higher doses of theaflavin-3-gallate showed comparable effect as fluoxetine and imipramine hydrochloride in terms of its antidepressant action. Similarly, in tail suspension test, we observed similar results as theaflavin-3-gallate considerably reduced the immobility time especially at higher doses. These results show that theaflavin-3-gallate has a dose-dependent antidepressant-like effect that is equivalent to well-known antidepressant drugs.
Conclusion
Theaflavin-3-gallate exhibits significant anti-depressant effect both in vitro and in vivo.
Ethical Issue
The use of experimental animals was performed in accordance with the National Institute of Health Guide for the Care and Use of Laboratory Animals (publication No. 80-23, revised 1996). The use of experimental mice was approved by the Animal Experimentation Ethics Committee of the China-Japan Union Hospital of Jilin University, Changchun, China.
References
Alley MC, Scudiero DA, Monks A, Hursey ML, Czerwinski MJ, Fine DL, Abbott BJ, Mayo JG, Shoemaker RH, Boyd MR. Feasibility of drug screening with panels of human tumor cell lines using a microculture tetrazolium assay. Cancer Res. 1988; 48: 589-601.
Baum RM. The darkness of depression. Chem Eng News. 2004; 82: 5.
Berton O, Nestler EJ. New approaches to antidepressant drug discovery: Beyond monoamines. Nat Neurosci. 2006; 7: 137-51.
Bahuguna A, Khan I, Bajpai VK, Kang SC. MTT assay to evaluate the cytotoxic potential of a drug. Bangladesh J Pharmacol. 2017; 12: 115-18.
Bhutani MK, Bishnoi M, Kulkarni SK. Anti-depressant like effect of curcumin and its combination with piperine in unpredictable chronic stress induced behavioral, biochemical and neurochemical changes. Pharm Biochem Behav. 2009; 92: 39-43.
Butterweck V, Jürgenliemk G, Nahrstedt A, Winterhoff H. Flavonoids from Hypericum perforatum show antidepressant activity in the forced swimming test. Planta Med. 2000; 66: 3-6.
Callaghan CK, Kelly AM. Neurotrophins play differential roles in short and long-term recognition memory. Neurobiol Learn Mem. 2013; 104: 39-48.
Cotter D, Mackay D, Chana G, Beasley C, Landau S, Everall IP. Reduced neuronal size and glial cell density in area 9 of the dorsolateral prefrontal cortex in subjects with major depressive disorder. Cereb Cortex. 2002; 12: 386-94.
Cotter D, Mackay D, Landau S, Kerwin R, Everall I. Reduced glial cell density and neuronal size in the anterior cingulate cortex in major depressive disorder. Arch Gen Psychiatry. 2001; 58: 545-53.
Duman RS. Pathophysiology of depression: The concept of synaptic plasticity. Eur Psychiatry. 2002; 17: 306-10.
Eckeli AL, Dach F, Rodrigues ALS. Acute treatment with GMP produces antidepressant-like effects in mice. Neuro Rep. 2000; 11: 1839-43.
Ernst E. Herbal remedies for depression and anxiety. Adv Psychiatr Treat. 2007; 13: 312-16.
Gupta S, Kashyap P, Asad M, Chattopadhyaya I, Dahiya R. Anti-depressant activity of Nyctanthes arbortristis in mice. Bangladesh J Pharmacol. 2016; 11: 634-45.
Kim JJ, Yoon KS. Stress: Metaplastic effects in the hippo-campus. Trends Neurosci. 1998; 21: 505-09.
Kou J, Lu C, Wang J, Chen Y, Xu Z, Varma RS. Selectivity enhancement in heterogeneous photocatalytic transformations. Chem Rev. 2017; 117: 1445-514.
Lee BH, Kim YK. The roles of BDNF in the pathophysiology of major depression and in antidepressant treatment. Psychiatry Investig. 2010; 7: 231-35.
Leung LK, Su Y, Chen R, Zhang Z, Huang Y, Chen ZY. Theaflavins in black tea and catechins in green tea are equally effective antioxidants. J Nutr. 2001; 131: 2248-51.
Li YF, Liu YQ, Yang M, Wang HL, Huang WC, Zhao YM, Luo ZP. The cytoprotective effect of inulin-type hexasaccharide extracted from Morinda officinalis on PC12 cells against the lesion induced by corticosterone. Life Sci. 2004; 75: 1531-38.
Mahmoudi M, Ebrahimzadeh MA, Dooshan A, Arimi A, Ghasemi N, Fathiazad F. Antidepressant activities of Sambucus ebulus and Sambucus nigra. Eur Rev Med Pharmacol Sci. 2014; 18: 3350-53.
Mills E, Montori VM, Wu P, Gallicano K, Clarke M, Guyatt G. Interaction of St John's wort with conventional drugs: Systematic review of clinical trials. BMJ. 2005; 329: 27-30.
Moklas MAM, Nurul Raudzah AR, Taufik Hidayat M, Sharida F, Farah Idayu N, Zulkhairi A, Shamima AR. A preliminary toxicity study of mitragynine, an alkaloid from Mitragyna speciosa Korth and its effects on locomotor activity in mice. Adv Med Dent Sci. 2008; 2: 56-60.
Muralidharan P, Balamurugan G, Babu V. Cerebroprotective effect of Glycyrrhiza glabra Linn. root extract. Bangladesh J Pharmacol. 2008; 4: 60-64.
Oliveira SL, Pillat MM, Cheffer A, Lameu C, Schwindt TT, Ulrich H. Functions of neurotrophins and growth factors in neurogenesis and brain repair. Cytometry A. 2013; 83: 76-89.
Pan MH, Lai CS, Ho CT. Anti-inflammatory activity of natural dietary flavonoids. Food Funct. 2010; 1: 15-31.
Pathak L, Agrawal Y, Dhir A. Natural polyphenols in the management of major depression. Expert Opin Investig Drugs. 2013; 22: 863-80.
Pittler MH, Ernst E. Kava extract for treating anxiety. Cochrane Database of Syst Rev. 2003; 1: CD003383.
Porsolt RD, Bertin A, Jalfre M. Behavioral despair in mice: A primary screening test for antidepressants. Arch Int Pharmacodyn Ther. 1977; 229: 327-36.
Rabiei Z, Gholami M, Rafieian-Kopaei M. Antidepressant effects of Mentha pulegium in mice. Bangladesh J Pharmacol. 2016; 11: 711-15.
Rajkowska G. Histopathology of the prefrontal cortex in major depression: What does it tell us about dysfunctional monoaminergic circuits. Prog Brain Res. 2000; 126: 397-412.
Rocha FF,Lima Landman T, Souccar C, Tanae MM, De Lima TC, Lapa AJ. Antidepressant-like effect of Cecropia glazioui Sneth and its constituents: In vivo and in vitro characterization of the underlying mechanism. Phytomedicine 2007; 14: 396-402.
Salehi A, Setorki M. Effect of Hyssopus officinalis essential oil on chronic stress-induced memory and learning impairment in male mice. Bangladesh J Pharmacol. 2017; 12: 448-54.
Setorki M, Hooshmandi Z. Neuroprotective effect of Ziziphus spina-christi on brain injury induced by transient global cerebral ischemia and reperfusion in rat. Bangladesh J Pharmacol. 2017; 12: 69-76.
Slater TF, Sawyer B, Sträuli U. Studies on succinate-tetrazolium reductase systems: III. Points of coupling of four different tetrazolium salts III. Points of coupling of four different tetrazolium salts. Biochim Biophys Acta. 1963; 77: 383-93.
Steru L, Chermat R, Thierry B, Simon P. The tail suspension test: A new method for screening antidepressants in mice. Psychopharmacology 1985; 85: 367-70.
van de Loosdrecht AA, Nennie E, Ossenkoppele GJ, Beelen RH, Langenhuijsen MM. Cell mediated cytotoxicity against U 937 cells by human monocytes and macrophages in a modified colorimetric MTT assay: A methodological study. J Immunol Methods. 1991; 141: 15-22.
Xia X, Cheng G, Pan Y, Xia ZH, Kong LD. Behavioral, neurochemical and neuroendocrine effects of the ethanolic extract from Curcuma longa L. in the mouse forced swimming test. J Ethnopharmacol 2007; 110: 356-63.
Xu C, Luo L, Tan RX. Antidepressant effect of three traditional Chinese medicines in the learned helplessness model. J Ethnopharm. 2004; 91: 345-49.
Yu ZF, Kong LD, Chen Y. Antidepressant activity of aqueous extracts of Curcuma longa in mice. J Ethnopharmacol. 2002; 83: 161-65.
Zomkowsi ADE, Rosa AO, Lin J, Santos ARS, Calixto JB, Rodrigues ALS. Evidence for serotonin receptor subtypes involvement in agmatine antidepressant like-effect in the mouse forced swimming test. Brain Res. 2004; 1023: 256-63.
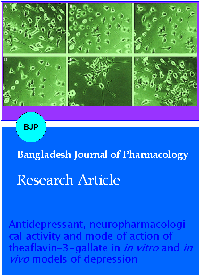